Five new ways to catch gravitational waves — and the secrets they’ll reveal

Observatories, experiments and techniques are being developed to spot ripples in space-time at frequencies that currently can’t be detected
In September 2015, a vibration lasting just one-fifth of a second changed the history of physics. It was the first direct detection of gravitational waves — perturbations in the geometry of space-time that move across the Universe at the speed of light.
Astronomers say it was like gaining a new sense — as if, until 2015, they had only been able to ‘see’ cosmic events, and now could ‘hear’ them, too. Since then, it has become almost a matter of daily routine to record the passage of gravitational waves at the two massive facilities of the Laser Interferometer Gravitational-wave Observatory (LIGO) in Louisiana and Washington state, along with their sibling Virgo observatory near Pisa, Italy.
The detection of gravitational waves has provided new ways to explore the laws of nature and the history of the Universe, including clues about the life story of black holes and the large stars they originated from. For many physicists, the birth of gravitational-wave science was a rare bright spot in the past decade, says Chiara Caprini, a theoretical physicist at the University of Geneva in Switzerland. Other promising fields of exploration have disappointed: dark-matter searches have kept coming up empty handed; the Large Hadron Collider near Geneva has found nothing beyond the Higgs boson; and even some promising hints of new physics seem to be fading. “In this rather flat landscape, the arrival of gravitational waves was a breath of fresh air,” says Caprini.
That rare bright spot looks set to become brighter.
All of the more than 100 gravitational-wave events spotted so far have been just a tiny sample of what physicists think is out there. The window opened by LIGO and Virgo was rather narrow, limited mostly to frequencies in the range 100–1,000 hertz. As pairs of heavy stars or black holes slowly spiral towards each other, over millions of years, they produce gravitational waves of slowly increasing frequency, until, in the final moments before the objects collide, the waves ripple into this detectable range. But this is only one of many kinds of phenomenon that are expected to produce gravitational waves.
LIGO and Virgo are laser interferometers: they work by detecting small differences in travel time for lasers fired along perpendicular arms, each a few kilometres long. The arms expand and contract by minuscule amounts as gravitational waves wash over them. Researchers are now working on several next-generation LIGO-type observatories, both on Earth and, in space, the Laser Interferometer Space Antenna; some have even proposed building one on the Moon1. Some of these could be sensitive to gravitational waves at frequencies as low as 1 Hz.
But physicists are also exploring entirely different techniques to detect gravitational waves. These strategies, which range from watching pulsars to measuring quantum fluctuations, hope to catch a much wider variety of gravitational waves, with frequencies in the megahertz to nanohertz range (see ‘Opening the window on gravitational waves’).
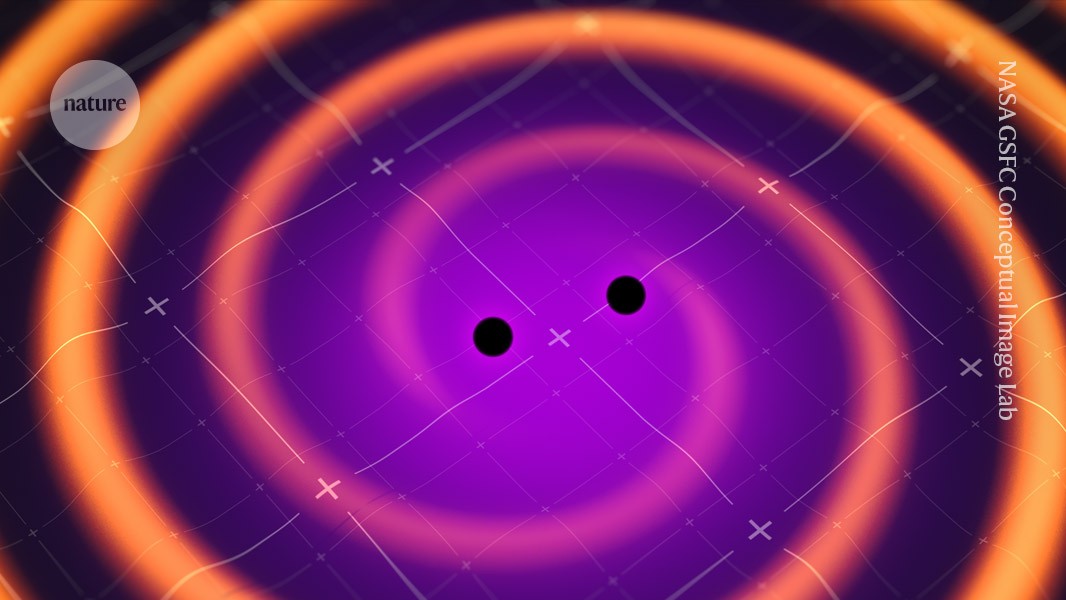
By broadening their observational window, astronomers should be able to watch black holes circling each other for days, weeks or even years, rather than just catching the last few seconds before collision. And they’ll be able to spot waves made by totally different cosmic phenomena — including mega black holes and even the start of the Universe itself. All this, they say, will crack open many remaining secrets of the cosmos.
Pulsar timing array: catching waves that last a decade
Last year, one viable alternative to interferometers entered the game.
Since the early 2000s, radio astronomers have been attempting to use the entire Galaxy as a gravitational-wave detector. The trick is to monitor dozens of neutron stars called pulsars. These spin on their axis hundreds of times per second while emitting a radio-frequency beam, producing what looks like a pulse of light on each turn.
Gravitational waves sweeping the Galaxy would change the distance between Earth and each pulsar, creating anomalies in detected pulsar frequencies from one year to the next. Observations of a collection or array of pulsars — called a pulsar timing array (PTA) — should be able to detect changes induced by gravitational waves with frequencies of just nanohertz, as might be produced by pairs of supermassive black holes, for example. It takes tens of years for successive crests of such waves to pass a given vantage point, meaning that tens of years of observations are needed to spot them.
In 2023, the PTA technique began to bear fruit. Four separate collaborations, in North America, Europe, Australia and China, unveiled tantalizing hints of a pattern expected from a random ‘stochastic background’ of gravitational waves that make Earth slosh around, probably caused by a cacophony of supermassive black-hole binaries, says astrophysicist Chiara Mingarelli at Yale University in New Haven, Connecticut.
The teams have not yet used the word ‘discovery’, because the evidence that each collaboration unveiled is not yet rock solid. But three of the groups — all but the Chinese one — are now pooling their data and conducting a joint analysis in the hope of getting to the ‘D’ word. This requires painstaking work, because each group processed its raw data in slightly different ways, and so it could take at least another year to get to publication, says Scott Ransom, an astrophysicist at the US National Radio Astronomy Observatory in Charlottesville, Virginia, and a senior member of the North American collaboration.
“In our current data, we almost certainly have the hints of individual supermassive black-hole binaries out there,” says Ransom. With each extra year of observation, they should get closer to resolving single black-hole pairs out of the cacophony, he adds. “Things are just going to get better and better.”
Microwave telescopes: spotting waves from the Big Bang
A year before LIGO’s 2015 detection, a team of cosmologists using a South Pole telescope called BICEP2 claimed to have spotted gravitational waves — not directly, but in the pattern of light called the cosmic microwave background (CMB), sometimes described as the afterglow of the Big Bang.
The BICEP2 claim turned out to be premature, but cosmologists are now doubling down on this idea. An array of telescopes much more powerful than BICEP2, called the Simons Observatory, is being set up on a mountaintop in northern Chile’s Atacama Desert. Some researchers are holding out hope for an even more powerful array called CMB-S4 (originally proposed to include 12 telescopes in Chile and at the South Pole) — although in May, plans for that project were put on hold because of the disrepair of the US South Pole base.
What cosmologists are looking for in the CMB is a specific ‘B mode’ pattern in the swirls of its polarization — the preferential directions in which the microwaves wiggle — that would have been imprinted by the passage of gravitational waves. The theory is that such waves should have been produced by inflation, a quick burst of exponential cosmic expansion thought to have happened around the time of the Big Bang2. Inflation would explain many of the Universe’s most striking properties, such as its flatness and how mass is distributed. The gravitational waves that inflation produced would have started at high frequencies, but would by now be at incredibly low frequencies of around 10−14 Hz.
Although inflation is a cornerstone of accepted cosmological theory, there’s no proof of it yet. The B-mode pattern would be the smoking gun and, moreover, would reveal the energy scales involved, which would be a first step towards understanding what powered inflation.
The problem is, no one knows whether that energy scale was large enough to have left a noticeable mark. “Inflation predicts the B modes, but we don’t know if it’s big enough to be detected,” says Marc Kamionowski, a theoretical astrophysicist at Johns Hopkins University in Baltimore, Maryland. But if the leading models are right, either the Simons Observatory or CMB-S4 should eventually find it, he says.
Atom interferometry: closing the gap
Although many of these projects push gravitational-wave science towards lower frequencies, they leave a crucial gap just below 1 Hz.
Detecting such frequencies could reveal mergers of black holes much more massive than those seen by LIGO (which spots waves from collapsing stars that weigh at most a few tens of solar masses). “This is an unexplored region, but it could be populated with lots of black holes,” says Caprini.

Jason Hogan (left) and Mark Kasevich work on an atom interferometer — a device that could reveal mergers of black holes much more massive than those seen by current laser interferometers.Credit: L.A. Cicero and Stanford University
A nascent technique could come to the rescue, according to physicist Oliver Buchmüller at Imperial College London. “Atom interferometry sits in that gap which we currently cannot explore with any other technology,” he says. An atom interferometer is a vertical high-vacuum pipe in which atoms can be released and allowed to fall under gravity. As they do so, physicists tickle the atoms with laser light to toggle them between an excited and a relaxed state — the same principle used by atomic clocks. “We’re trying to push this atomic-clock technique to what’s ultimately possible,” says Jason Hogan, a physicist at Stanford University in California.
To detect gravitational waves, physicists plan to drop two or more sets of atoms at different heights inside the same vertical pipe, and to measure the time it takes for a laser pulse to travel from one set of atoms to the next, says Hogan. The passage of gravitational waves would result in light spending either slightly less or slightly more time travelling between them — a variation smaller than one part in 100 billion billion.
Pioneering experiments at Stanford University have developed atom interferometers with 10-metre drops, but detecting gravitational waves would require devices at least 1 kilometre in height, which could be installed in a mine shaft, say, or even in space. As a first step, several groups around the world are planning to build 100-m atom interferometers as test beds. One such facility, called MAGIS-100, is already under construction in an existing shaft at the Fermi National Accelerator Laboratory outside Chicago, Illinois, and is scheduled for completion in 2027.
Desktop detectors: pushing the frequency up
Other researchers are exploring ways of detecting gravitational waves with much, much smaller (and cheaper) detectors — including some that could fit on a desktop. These are designed to watch for extremely high-frequency gravity waves. Known phenomena probably don’t produce such waves, but some speculative theories do predict them.
The Levitated Sensor Detector (LSD) at Northwestern University in Evanston, Illinois looks like a toy LIGO: it bounces lasers between pairs of mirrors just 1 metre apart. The LSD is a prototype for a new type of instrument designed to sense gravitational waves using resonance: the same principle by which even little pushes can make a child on a swing go higher and higher if they are timed just right3.
In a vacuum inside each of the LSD’s arms, laser light suspends a particle just micrometres wide. As with an interferometer, the passage of gravitational waves will alternately elongate and compress the length of each arm. If the frequency of the gravitational waves resonates with that of the device, the lasers will then give many tiny kicks to the particle. The LSD can track the particle’s motion with a precision of femtometres, says Northwestern physicist Andrew Geraci, who is leading the project.
The LSD is designed to be sensitive to gravitational waves with frequencies of around 100 kHz. This prototype might already have a shot at detecting some, if the team can keep experimental noise in check — and provided that such waves exist. “Depending how optimistic you are, we may be able to measure a real signal in that band even with a 1-m instrument,” Geraci says. Future versions could be scaled up to 100-m-long arms, he adds, which would increase their sensitivity.
Theoretical physicist Ivette Fuentes at the University of Southampton, UK, has an idea for making an even smaller resonant detector. She aims to exploit sound waves in an exotic state of matter called a Bose–Einstein condensate (BEC) — a cloud of atoms kept at temperatures as low as a few millionths of a degree above absolute zero. If a gravitational wave passes through at a frequency that resonates with the sound wave, it can be detected. Because the act of looking for this signal destroys the BEC, a new flood of atoms needs to be released every second. The process might need to be repeated for months for a successful detection, Fuentes says.
In principle, a BEC-based detector could expand the search for gravitational waves to extremely high frequencies of 1 MHz or more — again, provided they exist. Fuentes says her scheme would require pushing BEC techniques just a little beyond the current state of the art. “I think the idea is very bold,” she says. Physicists have posited that high-frequency gravitational waves could reveal exotic physics that went on in the first second or so after the Big Bang. “We could use it to study the state of the Universe at very high energies,” says Caprini.
Quantum crystal: only takes a second
A final, more radical proposal for detecting gravitational waves involves putting objects in two places at once.
Sougato Bose, a physicist at University College London, has proposed a device in which a micrometre-sized diamond crystal is put in a superposition of two quantum states. In his scheme, the crystal’s two ‘personas’ would be pushed apart by as much as 1 metre and then brought together again — an extremely delicate procedure that has been compared to putting the nursery-rhyme character Humpty Dumpty back together after a fall. The passage of gravitational waves would make one persona travel further than the other when apart, putting them out of sync — in a measurable way — when reunited. The whole process would take around one second to complete, which would make the device sensitive to gravitational waves of around 1 Hz.
This idea is extremely ambitious: such quantum tricks have so far been shown to work only for objects the size of molecules, and no one has ever tested whether quantum weirdness can be pushed to such extremes. “Putting Humpty Dumpty back together has never been demonstrated for crystals,” says Bose.
But if the technique can be perfected, then table-top experiments such as this one could take gravitational-wave detection out of the hands of just a few large-scale labs. Together, these techniques could blow open the window on what can be seen. “The outlook is very positive,” says Caprini.
doi: https://doi.org/10.1038/d41586-024-02003-6
This story originally appeared on: Nature - Author:Davide Castelvecchi