Where do proteins go in cells? Next-generation methods map the molecules’ hidden lives

Spatial proteomics is helping biologists to uncover how cells work by mapping where proteins operate
The average human cell contains roughly 10,000 different proteins. Existing as several to millions of copies in each cell, proteins mediate all manner of tasks, including chemical transformations, communication and intracellular trafficking. To execute these crucial functions, each protein must cosy up with designated partners, says Emma Lundberg, a bioengineer at Stanford University in California. If you want to understand how cells function, you have to work out what proteins interact with — and where.
To coordinate the mass of moving parts, eukaryotic cells evolved membrane-bound organelles and other subcellular compartments that gather protein networks together in controlled environments, each with a distinct function. By working out the sites at which a protein operates, researchers can infer something about its job. “It’s like a person in a house: if you are in the kitchen or in your laundry room, I can make a pretty good guess of what you’re doing,” says Lundberg.
But, like people, proteins aren’t restricted to one place; as researchers such as Lundberg in the fast-growing field of spatial proteomics have discovered, more than half of our proteins have more than one hangout spot. Many have multiple functions and moonlight in different roles depending on their position. By the same token, cellular processes falter when proteins show up to the wrong job site. “It really shows that this notion of ‘one gene, one protein, one function’ is really not correct,” Lundberg says.
With advances in high-resolution imaging, mass spectrometry and machine-learning-assisted data analysis, spatial-proteomics researchers are mapping and tracking proteins with increasing detail at multiple scales, from subcellular compartments to tissues. The work is challenging and data-intensive, but findings have yielded insights into cellular biology and disease progression — not to mention, potential therapeutic strategies.
Cellular cartography
Proteomics is to proteins what transcriptomics is to RNA. By counting the RNA-based messages that the cell writes to direct its protein-making machinery, transcriptomics practitioners hope to deduce what the cell is doing. But the expression level of a given transcript doesn’t necessarily match the abundance of its corresponding protein. And cells often edit their transcripts and modify proteins after translation to create different variants, called proteoforms.
Enter proteomics, which provides concrete data to explain how the ‘parts list’ of possible proteins in our genes is assembled to build the cell types in the body. By mapping protein abundances to their physical locations, spatial proteomics highlights how those proteins — and cells — are organized in tissues. There are two basic approaches: the imaging method fluorescence microscopy and quantitative mass spectrometry. The methods are complementary, and two large-scale atlas projects demonstrate the power of using them together.
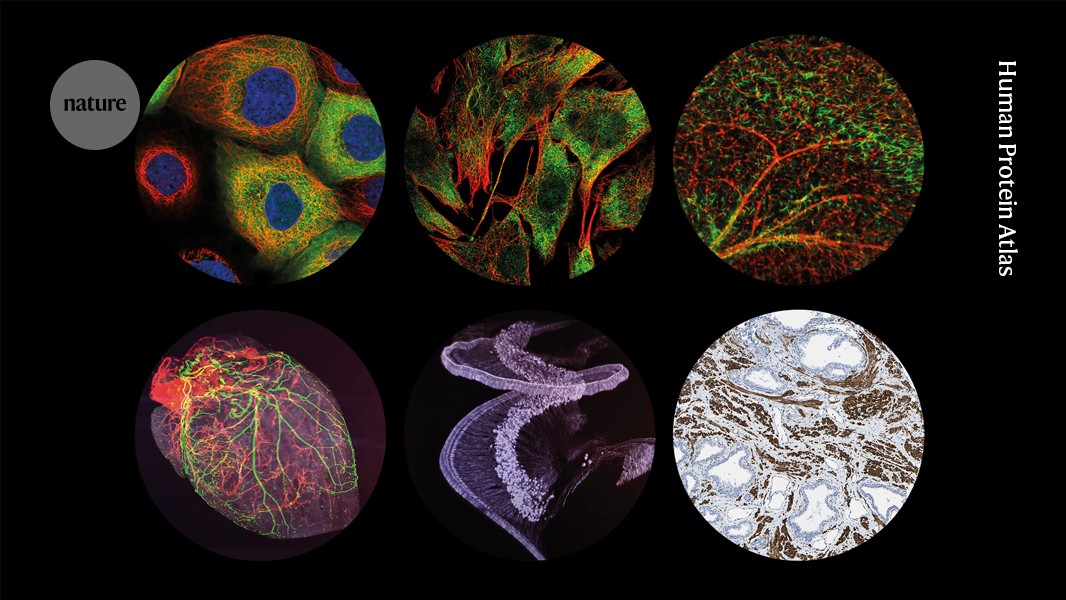
A tissue sample is prepared for highly multiplexed proteoform imaging.Credit: Lisa La Vallee
In 2017, Lundberg and her collaborators used a mix of both methods to build a subcellular atlas of more than 12,000 proteins in 22 cell types called the Cell Atlas, which is part of the Sweden-based Human Protein Atlas project1. The team stained a library of cells with fluorescently labelled antibodies for each target protein alongside markers for notable structures, such as the nucleus and mitochondria. These cellular landmarks helped the researchers to assign each protein to one or more of 30 subcellular locations, from major organelles to more esoteric options, such as cell junctions and lipid droplets.
Immunofluorescence works well when researchers know what proteins they are looking for and have suitable antibodies that can tag them, says Kathryn Lilley, a biochemist at the University of Cambridge, UK. Newer methods that combine repeated cycles of staining and imaging — such as co-detection by indexing (CODEX) and iterative bleaching extends multiplexity (IBEX) — can visualize tens to hundreds of labelled proteins in a single sample. But even these multiplexed methods overlook most of the proteome. Mass spectrometry can help to complete the picture, Lilley says.
Lilley and her colleagues developed a suite of mass-spectrometry-based techniques for subcellular mapping that are broadly called ‘localization of organelle proteins by isotope tagging’ (LOPIT). To validate the Cell Atlas’s image-based assignments, the researchers applied a variant called hyperLOPIT that works by carefully breaking cells open, separating their contents across a density gradient and digesting the proteins from each sample using enzymes. Next, they label each sample with a different isotope tag, recombine them and analyse the resulting mixture using tandem mass spectrometry — a quantitative approach that allows them to identify, quantify and assign thousands of proteins back to their original subcellular compartments.
The team used these and other methods to demonstrate that more than half of the proteins in human cells could be found in more than one location, and a subset could be found in at least three. About one-third of the proteins were present in every cell type, suggesting that they have generic ‘housekeeping’ roles that keep our cells running.
In 2022, Manuel Leonetti at the Chan Zuckerberg Biohub in San Francisco, California, working with Matthias Mann at the Max Planck Institute of Biochemistry in Munich, Germany, and their colleagues on the OpenCell project generated a library of 1,310 lines of human embryonic kidney cells, each of which fused a genetically encoded fluorescent protein tag to a different protein target. The team then used fluorescence microscopy to manually annotate the distribution of the tagged protein in living cells of each type and to train a machine-learning algorithm to carry out the same assignments. At the same time, the genetically encoded tags provided an antigen with which Mann’s team could perform immunoprecipitation-coupled mass spectrometry experiments. These allow the researchers to grab hold of a protein and its interaction partners and then train a second machine-learning algorithm to systematically build up a ‘social network’ for these proteins. Users can explore each protein’s whereabouts and known associates on the OpenCell web portal.
Take, for example, FAM241A, an ‘orphan’ protein of previously unknown function. When Leonetti and his colleagues looked at cells engineered to express FAM241A with a fluorescent tag, they found it in the endoplasmic reticulum, an organelle that, among other things, affixes chemical modifications to proteins. From those snapshots, the project’s machine-learning algorithm predicted that FAM241A might interact with two enzymes from the OpenCell library that add a specific type of sugar molecule to proteins. Further investigation confirmed that FAM241A is part of the same pathway, revealing its function2.
Moving molecules
Living cells are dynamic, ceaselessly building and breaking biomolecules, and shuffling them from one location to another. Detailed descriptions of where proteins typically congregate — and how they move from place to place — can help researchers to understand the significance of protein movements when cells are stressed or during disease.
In one study3, for instance, Lilley and her colleagues measured how the proteome of lung cancer cells responds to radiotherapy — a common treatment for this disease. Using a LOPIT-based workflow, the team measured the proteomes of cultured lung cancer cells exposed to X-rays and those of untreated controls. Of the several hundred proteins that differed between the two conditions, most changed in either abundance or location, but rarely in both, Lilley says. Focusing on those proteins that seemed to migrate after exposure to radiation, the researchers identified several key players in an iron-dependent cell-death pathway called ferroptosis that might contribute to radiotherapy resistance in some cancers. Although the work is preliminary, the pathway is a potential therapeutic target, Lilley says.
Similarly, Mikko Taipale, a functional geneticist at the University of Toronto in Canada, and his colleagues coaxed human cell cultures to produce 3,448 fluorescently tagged proteins in which a single-letter alteration to the coding sequence changes one amino acid for another. Such ‘missense mutations’ can sometimes disable a protein, he notes; at other times, “it leads to this rewiring of cellular networks so that the protein ends up in the wrong place and interacts with the wrong partners”. In this case, manual analysis of the microscopy data showed that 16% of variants that were known or likely to be pathogenic also congregated in the wrong place in cells, suggesting a link between protein location and disease4. “I don’t think I would have guessed that it’s that common,”Taipale says.
In fact, misplaced proteins in cancer and neurodegenerative disease “seems sufficiently widespread that an approach to fixing that mislocalization would be quite powerful”, says Stanford chemical biologist Steven Banik. Scientists have developed tools to study these processes either by reuniting estranged interaction partners5 or by sequestering crucial components in distant parts of the cell6. Now, they are attempting to exploit this information for therapeutic benefit.
Banik and his colleagues, for instance, have created a chemical ‘tow hitch’ called targeted relocalization-activating molecules (TRAMs) that latch on to a shuttle protein at one end and a designated cargo on the other7. In one demonstration of this technology, Banik’s team used an RNA-binding protein called FUS as cargo. This protein is normally present in the nucleus but can form toxic granules in the cytoplasm of neurons in some cases of the neurodegenerative condition motor neuron disease (amyotrophic lateral sclerosis). In cells that are manipulated to express both the faulty form of FUS and a protein that shuttles cargo from the cytoplasm to the nucleus, treatment with the matching TRAM effectively dissolved the troublesome granules by carting away the errant variants. With further development, Banik is hopeful that such molecular tools will uncover the rules that govern logistical networks in cells and even serve as medicinal compounds themselves.
Spatial conundrum
Despite these advances, significant knowledge gaps remain — even to seemingly obvious questions. For instance, just how big is the human proteome? Between variations in gene splicing, post-translational modification and folding, every protein-coding gene can give rise to multiple proteoforms, each of which might have a different function and location, says Neil Kelleher, a protein biochemist at Northwestern University in Evanston, Illinois.
To survey the proteoform landscape of cells and tissues, Kelleher and his collaborators combined an existing imaging system called nanospray desorption electrospray ionization (nano-DESI) and a new instrument for individual ion mass spectrometry, which is being commercialized by biotechnology company Thermo Fisher Scientific. Kelleher and his collaborators call the resulting platform ‘proteoform imaging mass spectrometry’ (PiMS); it can measure hundreds of proteoforms at the tissue level with a resolution of the order of a few cells. In one analysis of ovarian cancer biopsies, Kelleher and his colleagues identified 303 proteoforms that were expressed differently in healthy tissue and tumours8. Now, they are drilling down to the scale of isolated organelles such as nuclei.

A section of brain hippocampus from an individual with Alzheimer’s disease highlights proteins in different cell types: neurons (orange, magenta and green), astrocytes (cyan) and microglia (yellow). Amyloid-β plaques are in red and nuclei are in blue.Credit: Emma Lundberg Lab
Enjoying our latest content?
Login or create an account to continue
- Access the most recent journalism from Nature's award-winning team
- Explore the latest features & opinion covering groundbreaking research
or
Sign in or create an accountNature 640, 556-560 (2025)
doi: https://doi.org/10.1038/d41586-025-01045-8
This story originally appeared on: Nature - Author:Ariana Remmel